The use of biophysical methods in the hit-to-lead process
Posted: 22 June 2021 | Dr Thomas Schubert (2bind) | No comments yet
Optimisation of a hit towards a lead happens in a variety of ways, as a lead needs to meet various criteria such as activity, affinity to the target, selectivity, solubility, permeability or metabolic stability to become a promising candidate. A balance between in vitro profiles and pharmacokinetic attributes has to be achieved to increase the chances for successful drug development. Biophysical methods are used to address the in vitro binding parameters of affinity, kinetics, stoichiometry and thermodynamics, but also the structural information of the compound‑target interactions. Dr Thomas Schubert describes the current state-of-the-art and emerging biophysical methods applied in hit-to-lead programmes.
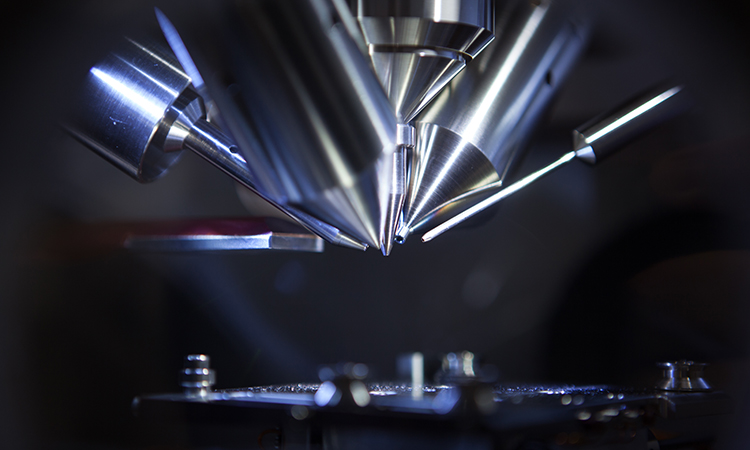
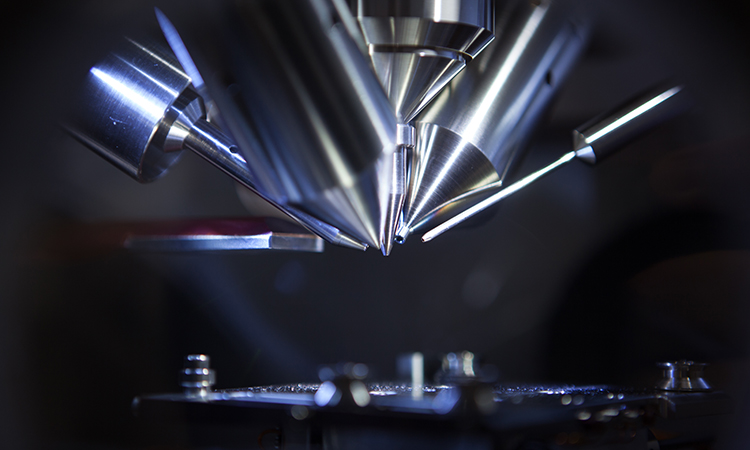
Since the first use of biophysical methods in the 1990s, biophysical assays have become increasingly important in the different steps of the complex drug discovery and development process. With the latest improvements in throughput, biophysical screening formats are now already used in the initial hit identification or primary screening steps. Traditionally, however, biophysical assay formats were mostly applied in the hit validation/conformation steps and in the hit-to-lead phase.1-3
In this phase – also called lead generation phase – biophysical, structural and biochemical data as well as data on cellular activity and absorption, distribution, metabolism and excretion (ADMET) data of a hit-hit series are studied, setting the basis for the optimisation of a hit towards a lead (lead optimisation).4 Systematic structure‑activity‑relationship (SAR) investigations in combination with medicinal chemistry approaches enable this optimisation step.
Biophysical assay formats assist hit-to-lead programmes by providing answers to important questions such as:
- Do the medicinal chemistry-optimised hits still bind to the target?
- Does the medicinal chemistry optimisation impact the affinity, kinetics or thermodynamics to the target?
- Do the medicinal chemistry-optimised hits influence the stability of the target – and if yes, how?
- Is the stoichiometry of the interaction modified by medicinal chemistry optimisations?
- Do the optimised hits still bind to the same site as the originator(s)?
- What does the structure of the complex of target and optimised hit(s) look like?
In this article, exemplary state-of-the art and emerging biophysical methods are presented that can help to find the answers for the above‑mentioned questions in SAR investigations.
Technologies supporting SAR investigations with structural information
X-ray crystallography: studying the structure of the target in presence and absence of a ligand
With X-ray crystallography, structural information is gained by analysis of crystals (protein alone) or co‑crystals (protein target in complex with compound).
Nuclear magnetic resonance (NMR) spectroscopy: studying the structure of the target in presence and absence of a ligand
In NMR, a broad spectrum of radio frequencies is applied to molecules placed in a magnetic field. Atomic nuclei will resonate with their own specific frequencies. NMR spectra can be extracted from these frequencies and structural information can be obtained. Different NMR measurement modes (ligand-observed and protein-observed) help SAR programmes with information ranging from binding affinity to information on binding mode and binding specificity.6 Additionally, compound solubility and integrity can be studied using NMR.3
Technologies supporting SAR investigations with binding parameters of the target-compound interaction
Native mass spectrometry (nMS): studying binding and interaction stoichiometry
Surface plasmon resonance (SPR): studying kinetics and binding affinities
SPR is when a polarised light beam hits a metal layer (commonly a gold film) at the interface of two media with different refractive indices. Kinetic parameters (association rate kon and dissociation rate koff) as well as steady-state affinity (Kd) can be calculated from changes in the refractive index upon binding of an interaction partner (analyte) to an immobilised partner (ligand) on the metal layer.8 Furthermore, thermodynamic parameters can be estimated from experimental repeats at different constant temperatures.
Grating coupled interferometry (GCI): studying kinetics and binding affinities
In line with SPR, GCI measures changes in the refractive index above a sensor surface. However, GCI is a waveguide-interferometry method, in which the light beam required for creating the evanescent field above the sensor surface is coupled with a reference beam into a waveguide below the sensor. This beam coupling leads to specific interference patterns. Once an analyte binds to the immobilised ligand, the refractive index change translates to a phase shift in the light beam. This generates a high-resolution, time-dependent and robust interference pattern, subsequently converted to the classical kinetic sensorgrams as in SPR.9 As for SPR analyses, repeats of GCI experiments at different temperatures allow for determination of thermodynamic parameters.
Biolayer interferometry (BLI): studying kinetics and binding affinities
BLI is another waveguide technology that analyses interference patterns of white light reflected from two optical layers on a sensor tip. One internal reference layer is located inside the tip and one layer at the interface between the tip and the surrounding liquid phase.
SwitchSENSE: studying kinetics, binding affinities and conformational changes
The SwitchSENSE technology monitors voltage‑driven movement of DNA nano‑levers attached to a sensor surface. Usually, such a nano‑lever carries one of the interaction partners by direct, covalent attachment. Binding of the other partner affects the hydrodynamic friction of the nano-lever – hence its movement on the sensor surface – which can be monitored through time-resolved single photon counting. Kinetic parameters (kon and koff) and steady-state affinity (Kd) can be extracted as well as conformational changes.11 Furthermore, thermodynamic parameters can be estimated by analyses at different temperatures.
MicroScale thermophoresis (MST): studying binding affinities
Measuring angles and intensities of these patterns leads to electron density maps that can then be converted into three‑dimensional (3D) structures with atomic resolution”
The optical MST method is based on the combined signal generated by the Temperature-Related Intensity Change (TRIC) effect of fluorescent molecules and their directed movement along temperature gradients (thermophoresis). The TRIC effect depends on changes of the fluorescence spectrum of the dye used for labelling the target protein, which can occur from ligand binding in close proximity to the dye or from conformational changes of the target protein that result from ligand binding. The thermophoretic component of the MST-signal varies with three key molecular features of the target-ligand entity that change upon binding: molecular size, molecular charge and change in the hydration shell of the molecules. Information on steady-state binding affinity (Kd) can be directly obtained from a ligand titration. By variation of assay temperatures, thermodynamics can also be determined. Measurements in bioliquids such as serum and cell lysates are possible.12
Isothermal titration calorimetry (ITC): rtudying thermodynamics, stoichiometry and binding affinities
Heat released or consumed during a molecular binding event is directly measured in ITC. This calorimetric technology offers high information content. Besides thermodynamic parameters such as ΔH, ΔS and ΔG, the equilibrium binding affinity (Kd) and interaction-stoichiometry can be determined from the same experiment.13 Recent developments even allow the study of kinetics using ITC.
Thermal shift assays (TSA): studying the influence of ligands on protein stability
Differential scanning fluorimetry (DSF) measures the temperature at which a protein unfolds by either utilising a fluorescent probe binding to exposed hydrophobic surfaces (classical DSF with dyes such as Sypro Orange) or using intrinsic fluorescence of a protein to monitor its unfolding (nanoDSF).14 The conformational stability of a protein is described by its unfolding transition midpoint (Tm), which is the point where half of the protein molecules in the solution are unfolded. Binding of a compound to a protein can be observed with a shift in Tm. Further information on the influence of a drug on protein stability, like protein aggregation or precipitation, can be obtained with specific nanoDSF assay setups.
Conclusion
Differential scanning fluorimetry (DSF) measures the temperature at which a protein unfolds”
In vitro binding parameters are a central building block of a promising lead profile. Still, biophysical data are often criticised because of their collection in artificial environments far off the reality in a cell/tissue/organism. Latest technological developments now push biophysics closer to the in vivo situation. Cellular thermal shift assays (CETSA) for example, allow for the study of target engagement in cells and tissues by monitoring thermal stability of a target in the presence of a compound in a cellular sample. MST and GCI enable the determination of binding affinity of a lead in bioliquids such as serum or cell lysates, containing potential hit off-targets or co-factors and other interaction partners of the target. Ranking of hits/leads according to their target affinity in selected bioliquids can assist SAR investigation by providing the information required for decisions: which lead should be prioritised or which lead modification should be explored. Latest reports also indicate a connection between in vitro kinetics of a lead/target interaction and pharmacokinetic and pharmacodynamic data.
About the author
Dr Thomas Schubert has over 10 years of experience in the use of biophysical technologies to study molecular interactions and is Chief Executive Officer of 2bind. He received his PhD in 2012, with a research focus on novel regulatory epigenetic mechanisms based on small RNAs. Thomas has also co-authored over 40 publications in international scientific journals.
References
- Holdgate G, Embrey K, Milbradt AG, Davies G. Biophysical methods in early drug discovery, ADMET & DMPK 7(4) (2019) 222-241
- Genick CC, Wrigth SK, Biophysics: for HTS hit validation, chemical lead optimization, and beyond. Expert Opin Drug Discov. 2017 Sep;12(9):897-907
- Renaud J, Chung C, Danielson U, et al. Biophysics in drug discovery: impact, challenges and opportunities, Nat Rev Drug Discov 2016 Oct;15(10):679-98.
- Hughes J, Rees S, Kalindjian S, Philpott K, Principles of early drug discovery, Br J Pharmacol 2011 Mar;162(6):1239-49.
- Maveyraud L, Mourey L. Protein X-ray Crystallography and Drug Discovery, Molecules. 2020 Feb 25;25(5):1030
- Li Y, Kang C. Solution NMR Spectroscopy in Target-Based Drug Discovery, Molecules. 2017 Aug 23;22(9):1399
- Hannah V, Atmanene C, Zeyer D, et al. Native MS: an ‘ESI’ way to support structure and fragment-based drug discovery, Future Med Chem. 2010 Jan;2(1):35-50
- Olaru A, Bala C, Jaffrezic-Renault N, Aboul-Enein H, Surface plasmon resonance (SPR) biosensors in pharmaceutical analysis. Crit Rev Anal Chem. 2015;45(2):97-105.
- Patko D, Cottier K, Hamori A, Horvath R. Single beam grating coupled interferometry: high resolution miniaturized label-free sensor for plate based parallel screening. Opt Express 2012 Oct 8;20(21):23162-73.
- Concepcion J, Witte K, Wartchow C, et al. Label-free detection of biomolecular interactions using BioLayer interferometry for kinetic characterization. Comb Chem High Throughput Screen 2009 Sep;12(8):791-800.
- Knezevic J, Langer A, Hampel PA, et al. Quantitation of affinity, avidity, and binding kinetics of protein analytes with a dynamically switchable biosurface. J Am Chem Soc. 2012 Sep 19;134(37):15225-8.
- Susanne A I, Seidel I, Dijkman P, et al. Microscale thermophoresis quantifies biomolecular interactions under previously challenging conditions. Methods. 2013 Mar;59(3):301-15.
- Holdgate GA, Ward WH, Measurements of binding thermodynamics in drug discovery. Drug Discov Today. 2005 Nov 15;10(22):1543-50
- Baljinnyam B, Ronzetti M, Yasgar A, Simeonov A. Applications of Differential Scanning Fluorometry and Related Technologies in Characterization of Protein-Ligand Interactions. Methods Mol Biol 2020;2089:47-68.
Related topics
Analytical techniques, Crystallography, Drug Leads, Hit-to-Lead, Mass Spectrometry, Nuclear Magnetic Resonance (NMR), Spectroscopy, X-ray crystallography
Related organisations
Creoptix