Antibodies against viral disease: highlighting technologies behind COVID-19 antibody therapy
Posted: 4 June 2020 | Shalom Gurjar (OXGENE) | No comments yet
Amid the rush to develop an effective vaccine against COVID-19, antibody therapy has become a major strategy to ameliorate and treat cases of severe disease. Armed with the capacity to neutralise pathogens and orchestrate the immune system, promising antibody candidates for multiple viral indications, including HIV, continue to emerge. Technology has not only revolutionised their safety, but also their rapid development from recovered patients or immunised animals. Here, Shalom Gurjar explores developments in antibody therapies, specifically focusing on viral infections.
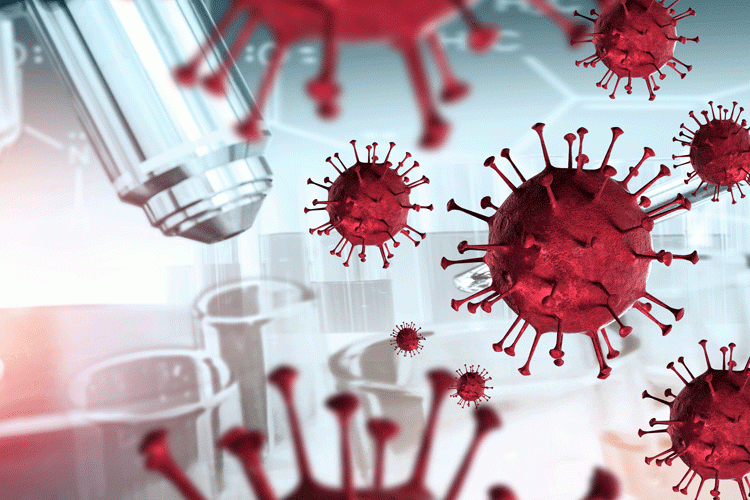
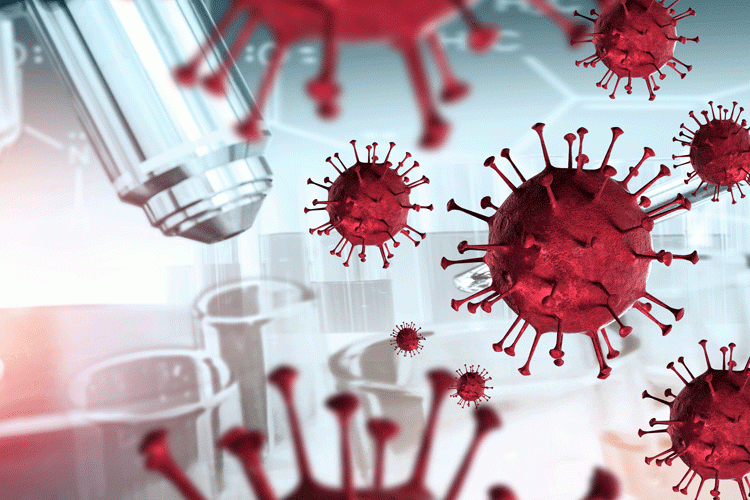
Over 100 years ago, Emil von Behring, in collaboration with Kitasato Shibasaburō, was awarded the first Nobel Prize in Medicine for the discovery of a treatment against diphtheria – a deadly infectious disease that had swept across the globe. The remarkable “antitoxin” constituted of polyclonal antibodies (pAbs) derived from vaccinated animals; this was later also applied to viral infections.1 Bioengineering has greatly enhanced the safety and efficacy of antibody therapies, converting them from animal products with strong immunogenicity to fully human proteins with limited side effects. Although palivizumab, a prophylactic monoclonal antibody (mAb) against respiratory syncytial virus, is the only approved mAb targeting a virus, numerous antibody cocktails against HIV, Zika and influenza are in development or clinical trials. A 2019 Ebola study punctuated their potential, reporting significantly improved patient mortality rates with two mAb‑based candidates (REGN-EB3 and mAb 114; 29 and 34 percent, respectively) compared to the anti‑viral remdesivir (53 percent).2 As serum from convalescent patients has recently become a first-line treatment for COVID-19, it is clear that antibody therapies are vital to help limit the severity of viral pandemics.
mAbs bind specifically and with high affinity to a range of antigen types. There are multiple mechanisms by which they neutralise or eradicate viruses, either operating directly or by harnessing the immune system (Figure 1). To achieve neutralisation, mAbs may target a surface glycoprotein via the receptor binding domain (RBD). These antibodies can also target the receptor or co-receptor itself, thereby hindering access via the virus’s normal route of infection. For instance, mAbs against both HIV-targeting receptors CD4 and co-receptor CCR5 are in clinical trials.3 The conformational change required to achieve membrane fusion and entry can also be obstructed. mAbs employ immune cells to eliminate agglutinated viruses and, compared to a single particle, these aggregates are an easier target for phagocytes to engulf.


Figure 1: mAbs prevent infection by neutralising the receptor binding domain (1), blocking cellular receptor(s) (2) and preventing target cell membrane fusion and endocytosis (3). Infection with newly formed virion particles is prevented by blocking egress (4). mAbs eradicate viruses by activating natural killer cells to eliminate infected cells (5) and agglutination (6) leading to phagocytosis by macrophages, with or without the complement system (7). Created with BioRender.com.
The contribution of Fc effector function is also vital to elimination. Assembly of the complement system can initiate phagocytosis and reportedly confers protective immunity against influenza and West Nile virus.4 Infected cells displaying viral proteins can be eradicated by Fc receptor‑bearing immune cells such as natural killer cells. For severe acute respiratory syndrome (SARS)‑causing coronaviruses, this may be mediated by macrophages and dendritic cells that defend mucosal-associated lymphoid tissues.5 Finally, mAbs can prevent egression of newly formed virion particles. This is utilised by influenza-specific mAbs against the haemagglutinin stalk region, which incidentally also inhibits neuraminidase activity.6
It is imperative that mAbs candidates are discovered and defined”
The passive transfer of human serum from convalescent donors is the most basic, but by no means least effective, form of antibody therapy. Apart from pre-screening donors, minimal manipulation is required and its application has been critical to curbing outbreaks of measles, mumps and influenza.7 This pAb treatment takes full advantage of the natural immune response.
Interestingly, levels of serum IgG against SARS‑CoV (2003 outbreak) are at their peak during the recovery stage.8 To assess the safety of passive transfer for COVID-19 treatment, there were over 2,000 co-ordinated studies in the US at the end of April.7 However, an obvious caveat to this strategy is availability, which may be overcome by a transgenic cattle platform developed by SAB Biotherapeutics to produce fully human pAbs. Cattle can be engineered to possess artificial human chromosomes and immunised with high levels of antigen, yielding an impressive 25g of antibody per litre. The potential of this technology has been exemplified with an anti-MERS-CoV antibody in a 2018 Phase I trial and SAB claims it may have a product ready as early as summer 2020.9 A more potent and consistent product is made by isolating the antibody fraction. Pioneered by Takeda and CSL Behring in collaboration with major blood product suppliers, the CoVIg-19 Plasma Alliance is an initiative to rapidly generate a single, large-scale product.10
It is clear that antibody therapies are vital to help limit the severity of viral pandemics”
There are around 60 labs developing mAbs against SARS-CoV-2 using approaches that bypass the complications surrounding donor‑derived blood products. Many groups make use of display technologies to screen antibody libraries derived from the B cells of recovered patients or immunised animals. Alternatively, synthetic antibody libraries that are highly diversified in the CDR H3 region, which is primarily responsible for antigen binding, are also used. Variable heavy and light chain genes are displayed as scFv or Fab by phage, bacteria, yeast or other mammalian cells and screened against a purified soluble antigen.11 Binders are isolated and re-cloned into the full IgG format, followed by affinity, activity and pharmacokinetic characterisation. Phage display has successfully identified potent antibodies against viral diseases including HIV, influenza and rabies.12 Furthermore, it has been used to screen for mAbs against SARS-CoV escape mutants, which evaded the human neutralising mAb CR3014. mAb CR3022 was selected based on complementarity to CR3014 and non-competitive epitope binding, followed by confirmation that escape mutants could not be generated. Interestingly, when combined, the benefit of targeting more than one epitope resulted in enhanced neutralisation.13
Single-cell reverse transcription PCR enables the construction of antibodies directly from FACS-selected antigen-specific B cells. Once expressed, mAbs are purified and validated. However, like display technology, defined purified antigen is required which may bypass candidates targeting novel epitopes. An alternative is to screen the mAbs directly from the supernatant of B cell clones that have been immortalised using cell culture techniques, such as hybridoma technology or more sophisticated Epstein-Barr virus (EBV) transformation. Supernatants can be screened for binding and/or neutralisation and PCR techniques employed to enable cloning and expression.14


Figure 2: Complex of the CR3022 mAb with the SARS-CoV-2 Spike structure (open state), modelled from recently determined structures, PDB:6VYB and PDB:6YLA.
For SARS-CoV, which also targets an epitope on Angiotensin-converting enzyme 2 (ACE2), the majority of neutralising mAbs were generated using phase display and EBV-transformed B cells. For SARS-CoV-2, depending on the technology, antibody discovery companies must select a suitable antigen to screen or generate mAbs. The coronavirus trimeric Spike (S) glycoprotein, specifically the RBD, is the prime target to prevent binding of SARS-CoV-2 to ACE2 or CD147, a recently identified additional receptor.15,16 Structural modelling identifies key residues at the antigen-antibody interface (Figure 2). This can inform vaccine design and steer affinity maturation.17 Of note, computer-generated docking simulation between the SARS-CoV-2 S protein and ACE2 has revealed that the aforementioned anti-SARS-CoV mAb CR3022 is a potential cross‑reacting candidate.18
At least three academic groups have published comprehensive reports on neutralising mAbs against the RBD, derived from transgenic mice or patient B cells. Staining of S protein-expressing cells confirmed specificity and ELISA or SPR-based competition assays were used to demonstrate ACE2 binding inhibition. In vitro, neutralisation of Vero cell infection with S protein pseudotyped lentivirus was confirmed, while one study reports in vivo results demonstrating reduced viral titres in a mouse model.19,20 One of the mAbs – fully human 47D11 from Utrecht University – was developed using Harbour transgenic mouse technology. Interestingly, 47D11 does not block ACE2 binding, as it instead binds to an epitope with the conserved core; potentially it blocks S protein-mediated cellcell fusion and thus infection of neighbouring cells. In line with the success of anti-Ebola cocktails, the authors highlight that 47D11 may be most potent in combination with other neutralising mAbs.21 Maplazumab, a humanised anti-CD147 mAb, is a candidate with an approved safety profile. Preprint results are promising, showing significantly improved recovery of a small cohort of hospitalised COVID-19 patients.22
Viral-associated pneumonia is a common manifestation of extreme COVID-19 that can send the immune system into overdrive. Orchestrated by mediators of inflammation, it can lead to respiratory and even organ failure. As a well-established strategy to ameliorate auto-immune disease and cytokine release syndrome, mAb therapy is currently in the spotlight. Several Phase II and III clinical trials are in progress, testing approved and development-stage mAbs against TNFα, Il-6, C5a and its receptor C5aR, and GMCSF, all of which have been found in high levels in patient plasma. Pre-print results are available from an independent hospital study in which anti‑IL-6 receptor Tocilizumab was administered. This‑demonstrated reduced mortality and admissions to intensive care.23
Vaccines remain the most powerful preventative strategy against viral diseases as they can confer life-long immunity, significantly in the form of the pAb response. Their development, however, typically takes many years, following which their safety and efficacy will need to be determined, although there is growing evidence that this stage may be fast-tracked. In addition to unwanted immunogenicity, antibody therapy in a viral context is not exempt from safety concerns. Antibody-mediated enhancement, observed in feline coronavirus infection, occurs when virion endocytosis is promoted by sub or non-neutralising levels of antibody. Their presence, likely the result of a related infection or in response to a vaccine, is linked to the progression of severe disease.24 However, current evidence suggests that correct dosing may be able to mitigate this. Furthermore, an obvious challenge to the widespread administration of antibodies is the high cost of production. This may be overcome by mass production in animals or prokaryotic systems. For now, it is imperative that mAbs candidates are discovered and defined.
About the author
Shalom Gurjar is a scientist at OXGENE working on a mammalian display platform to screen for mAbs against membrane proteins. Her PhD focused on assessing and modulating therapeutic mAbs and was carried out with the NIBSC and University of Manchester. During her BSc in Biochemistry she completed an internship targeting pathogenic tau in an Alzheimer’s disease context.
References
1. Winau F, Winau R. Emil von Behring and serum therapy. Microbes Infect. 2002;4(2):185–8.
2. New antibodies best ZMapp in Ebola trial. Nat Biotechnol. 2019;37(10):1105–1105.
3. Promsote W, DeMouth ME, Almasri CG, Pegu A. Anti-HIV-1 Antibodies: An Update. BioDrugs. 2020;34(2):121–32.
4. Ekiert DC, Bhabha G, Elsliger M-A, Friesen RHE, Jongeneelen M, Throsby M, et al. Antibody recognition of a highly conserved influenza virus epitope. Science. 2009;324(5924):246–51.
5. Liu L, Wei Q, Nishiura K, Peng J, Wang H, Midkiff C, et al. Spatiotemporal interplay of severe acute respiratory syndrome coronavirus and respiratory mucosal cells drives viral dissemination in rhesus macaques. Mucosal Immunol. 2016;9(4):1089–101.
6. Chen Y-Q, Lan LY-L, Huang M, Henry C, Wilson PC. Hemagglutinin Stalk-Reactive Antibodies Interfere with Influenza Virus Neuraminidase Activity by Steric Hindrance. J Virol. 2019;93:e01526–18.
7. Casadevall A, Pirofski L. The convalescent sera option for containing COVID-19. J Clin Invest. 2020;130(4):1545–8.
8. Cao W-C, Liu W, Zhang P-H, Zhang F, Richardus JH. Disappearance of antibodies to SARSassociated coronavirus after recovery. N Engl J Med. 2007;357(11):1162–3.
9. Beigel JH, Voell J, Kumar P, Raviprakash K, Wu H, Jiao J-A, et al. Safety and tolerability of a novel, polyclonal human anti-MERS coronavirus antibody produced from transchromosomic cattle: a phase 1 randomised, double-blind, single-dose-escalation study. Lancet Infect Dis. 2018;18(4):410–8.
10. CoVIg-19 Plasma alliance builds strong momentum through expanded membership and clinical trial collaboration. 2020 May 7 [cited 2020 May 14]. Available from: https://www.takeda.com/newsroom/newsreleases/2020/…
11. Tsuruta LR, Dos ML, Moro AM. Display Technologies for the Selection of Monoclonal Antibodies for Clinical Use. Antib Eng. 2017; DOI: 10.5772/ intechopen.70930.
12. Salazar G, Zhang N, Fu T-M, An Z. Antibody therapies for the prevention and treatment of viral infections. NPJ Vaccines. 2017;2(19).
13. ter Meulen J, van den Brink EN, Poon LLM, Marissen WE, Leung CSW, Cox F, et al. Human monoclonal antibody combination against SARS coronavirus: synergy and coverage of escape mutants. PLoS Med. 2006;3(7):e237.
14. Corti D, Lanzavecchia A. Efficient Methods To Isolate Human Monoclonal Antibodies from Memory B Cells and Plasma Cells. Microbiol Spectr. 2014;2(5).
15. Zhou G, Zhao Q. Perspectives on therapeutic neutralizing antibodies against the Novel Coronavirus SARS-CoV-2. Int J Biol Sci. 2020;16(10):1718–23.
16. Ulrich H, Pillat MM. CD147 as a Target for COVID-19 Treatment: Suggested Effects of Azithromycin and Stem Cell Engagement. Stem Cell Rev Rep. 2020;1–7.
17. Barderas R, Desmet J, Timmerman P, Meloen R, Casal JI. Affinity maturation of antibodies assisted by in silico modeling. Proc Natl Acad Sci U S A. 2008;105(26):9029–34.
18. Tian X, Li C, Huang A, Xia S, Lu S, Shi Z, et al. Potent binding of 2019 novel coronavirus spike protein by a SARS coronavirus-specific human monoclonal antibody. Emerg Microbes Infect. 2020;9(1):382–5.
19. Chen X, Li R, Pan Z, Qian C, Yang Y, You R, et al. Human monoclonal antibodies block the binding of SARS-CoV-2 spike protein to angiotensin converting enzyme 2 receptor. Cell Mol Immunol. 2020;1–3.
20. Wu Y, Wang F, Shen C, Peng W, Li D, Zhao C, et al. A noncompeting pair of human neutralizing antibodies block COVID-19 virus binding to its receptor ACE2. Science. 2020 May;eabc2241.
21. Wang C, Li W, Drabek D, Okba NMA, van Haperen R, Osterhaus ADME, et al. A human monoclonal antibody blocking SARS-CoV-2 infection. Nat Commun. 2020;11(1):2251.
22. Bian H, Zheng Z-H, Wei D, Zhang Z, Kang W-Z, Hao C-Q, et al. Meplazumab treats COVID-19 pneumonia: an open-labelled, concurrent controlled add-on clinical trial. medRxiv. 2020 Mar 21; Epub ahead of print.
23. Klopfenstein T, Zayet S, Lohse A, Balblanc J-C, Badie J, Royer P-Y, et al. Tocilizumab therapy reduced intensive care unit admissions and/or mortality in COVID-19 patients. Med Mal Infect. 2020 May 6; Epub ahead of print.
24. de Alwis R, Chen S, Gan ES, Ooi EE. Impact of immune enhancement on Covid-19 polyclonal hyperimmune globulin therapy and vaccine development. EBioMedicine. 2020 Apr 16; Epub ahead of print.
Related topics
Antibodies, Drug Development, Drug Discovery, Drug Targets, Immunology, Monoclonal Antibody, Research & Development, Therapeutics
Related conditions
Coronavirus, Covid-19, Epstein-Barr virus (EBV), Influenza, Middle East Respiratory Syndrome (MERS), Severe Acute Respiratory Syndrome (SARS), viral infections, West Nile virus, Zika virus
Related organisations
Thermo Fisher
Related people
Emil von Behring, Kitasato Shibasaburō