Advanced techniques and applications of LC-MS in small molecule drug discovery
Posted: 16 June 2016 | Baiwei Lin (Genentech Inc.), Chunang Gu (Genentech Inc.), Joseph Pease (Genentech Inc.), Kate Comstock (formerly of Roche) | No comments yet
A key process in drug discovery is the ability to identify new drug candidates by screening chemical libraries of small molecules, natural products or extracts against a biological target and selecting lead compounds with desirable therapeutic effects. Medicinal chemistry has the challenging role of not only ensuring that the chemical libraries are well suited for a broad range of disease targets but also of rapidly evolving lead molecules to drug candidates. With the implementation of parallel chemistry, proteomics, and absorption, distribution, metabolism and excretion (ADMET) profiling, the generation of new leads has increased tremendously…
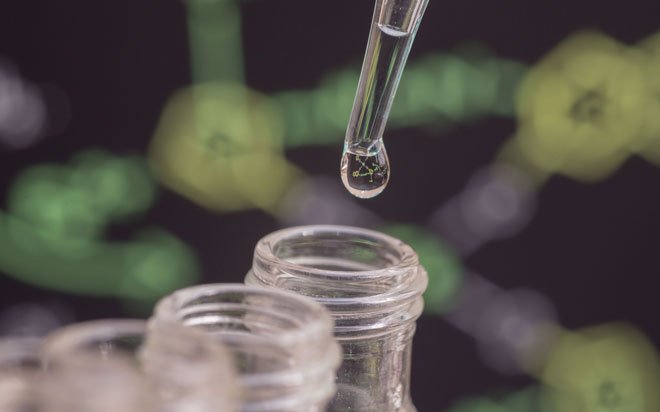
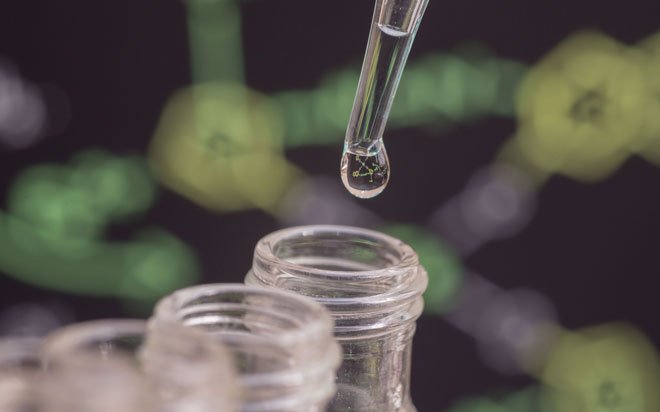
A key process in drug discovery is the ability to identify new drug candidates by screening chemical libraries of small molecules, natural products or extracts against a biological target and selecting lead compounds with desirable therapeutic effects1-6. Medicinal chemistry has the challenging role of not only ensuring that the chemical libraries are well suited for a broad range of disease targets but also of rapidly evolving lead molecules to drug candidates7- 11. With the implementation of parallel chemistry, proteomics, and absorption, distribution, metabolism and excretion (ADMET) profiling, the generation of new leads has increased tremendously.
Mass spectrometry (MS) has played an essential role in many stages of the drug discovery process to identify and characterise lead compounds – a process that is dynamic and involves multiple disciplines. The testing for quality control, characterisation, biological screening, physical properties and DMPK properties is crucial in order to evaluate drug candidate performance in efficacy, adsorption, desorption, metabolism, elimination and toxicity12-13. The process is lengthy, expensive and difficult, and involves iterative screening runs and chemistry drug designs to achieve optimal compound properties14-15. MS is an invaluable tool in this iterative process and is used to ensure high quality compound libraries, assess compound properties and to shorten the time needed to bring a lead compound to the clinic16.
Along with its hyphenated technologies such as liquid chromatography-MS (LC-MS) and gas chromatography-MS (GC-MS), MS enables the creation and development of high-throughput, qualitative and quantitative analytical methods used for drug discovery17. In combination, advances in LC and MS have enabled more efficient peak separation of complex mixtures employing multi – dimensional LC (2DLC) and more sensitive, higher resolution and ion mobility separation by MS as outlined below. In this review we briefly describe selected advanced LC-MS technologies applied to drug discovery chemistry, including compound ionisation, hyphenated systems, multidimensional systems, ion mobility spectrometry and high-resolution MS (HRMS) for quantitative analysis and impurity identification.
Mass spectrometry ionisation
Several ionisation techniques are used in MS. Each type of ionisation is favourable for particular types of molecules according to their molecular weight, structure and polarity. Figure 1 (page 00) briefly describes common ionisation techniques and applications18. Electron ionisation (EI) and chemical ionisation (CI) are mostly used for GC-MS19. Atmospheric pressure chemical ionisation and thermospray ionisation are used in LC-MS20-21. Electrospray (ESI) and ionspray (IS) ionisation are most efficient for polar molecules. ESI is widely used for both small molecules, such as synthetic organic compounds, and large biomolecules, such as proteins and peptides. Also, ESI is the most commonly-used ionisation source for LC-MS and can be readily configured for high-throughput screening assays22.
Other hyphenated techniques, such as GC-MS and, more recently, SFC-MS, are also routinely used for drug discovery. With EI or CI, GC-MS offers better detection and analytical results for very small and volatile compounds that are not easily detected by LC-MS using ESI23-26. Mass spectra from GC-MS provide reproducible fragment ions, which enables database dereplication and structural analysis. Supercritical fluid chromatography (SFC) employs carbon dioxide as its primary mobile phase at conditions above its critical pressure (7.39MPa or 1,071 psi) and temperature (304.25K or 31.10°C) to enable rapid separation and analysis of low-to-moderate molecular weight compounds (less than 1,000amu)27-28. SFC is commonly used for purification and separation of chiral compounds due to its fast separation times, which allows for rapid chiral column screening. SFC is easily scaled up to the preparatory level since minimal solvent is needed for separation which benefits enantiomers recovery. When combined with a mass spectrometer, the highly sensitive SFC-MS is used as a routine method for high throughput chiral and achiral analysis29-30.
The recently developed ambient ionisation technologies refer to a class of sampling ionisation techniques for direct ionisation of chemicals from samples in their raw or unprocessed ‘ambient’ state using spray, heat, plasma, high electric field or laser impact31. The potential value of ambient ionisation has been demonstrated by desorption electrospray ionisation and direct analysis in real time32. All of these technologies have shown that ambient ionisation can be used as a tool to provide efficient desorption and ionisation with minimal sample preparation for MS analysis.
Two dimensional LC-MS
LC-MS technologies provide effective means to analyse and characterise complex samples such as active pharmaceutical ingredients (APIs) by separating compounds of interest (COI) with LC in order to determine COI purities, identities and structures by UV diode array detection (DAD) and MS. Online two-dimensional HPLC (2DLC) has recently become commercially available and is being rapidly adopted by the pharmaceutical industry. 2DLC increases peak capacity and resolving power and is able to resolve complex mixtures that were previously not possible by one-dimensional HPLC33.
In 2DLC, fractions from the first dimension LC run are transferred to the second dimension LC for a second separation. A comprehensive 2DLC system is able to automatically transfer all fractions from first dimension separation to a holding loop or trapping column in preparation for the second dimension separation. Ideally, the holding time between the first and second dimension separations is very short. A common 2DLC method involves heart-cutting where only selected fractions from the first dimension separation are transferred to the second dimension; each fraction then undergoes a more selective and specific LC separation. This tandem LC x LC configuration allows analytical chemists to set up orthogonal conditions between the two dimensions, enabling superior separations and corresponding analytical results for a wide range of compounds.
According to the literature, 2DLC is applied in many areas including sample analysis in complex matrices, efficient profiling of complex mixtures, compound degradation studies, quantification of impurities that co-elute with API, and chiral compound separations34-38. Combining 2DLC with a mass spectrometer (2DLC-MS) is especially powerful for trace level impurity identification and quantification, minimising buffer and matrix interference to achieve higher MS sensitivity, online sample cleaning and dilution functions, and other benefits39-41.
A comprehensive 2DLC-MS system with a time-of-flight mass spectrometer has been reported by Liang Qu et al. for chemical constituent analysis from complex samples, in this case tripterygium glycosides tablets42. The group selected C8 and C18 columns for the first and second dimension chromatography, respectively, and integrated the 2DLC to the mass spectrometer to create a LC×LC-QTOF-MS system. With this high peak capacity separation and mass detection system, they detected 92 and 132 constituents from tripterygium glycosides tablets from different sources. This method provided powerful separation and detailed profiling information of the tablets to help with further studies, such as investigating toxic effects of the tablet constituents. The 2DLC-MS method has also been demonstrated as an effective purity assessment tool for the quality control and analysis of API samples. Alexander and Ma developed a comprehensive two-dimensional RPLC × RPLC method to address the specific problem of separating co-eluting impurities that were ‘hidden’ within the API peak in one-dimensional LC33. Venkatramani et al. have reported the use of analytical 2D RPLC × SFC for simultaneous achiral-chiral analysis of pharmaceutical compounds43. This orthogonal technique provides peak purity determination for trace level co-eluting impurities.
High resolution MS for quantitative analysis
MS-based quantitative analysis has traditionally been accomplished using tandem mass spectrometry (LC-MS/MS)44-46. This methodology is well established and is considered the ‘gold standard’ for MS quantitation due to its high selectivity and sensitivity. However, the workflow of this method is complex and time-consuming since it involves multiple operations of product ion selection, parent ion fragmentation and collision energy optimisation. Recently, researchers have reported using high-resolution accurate mass (HRMS) systems for quantitative analysis47-48. HRMS quantitation is relatively simple and does not rely on fragmentation for quantitative analysis. Mass selectivity on a HRMS system is dramatically increased by setting the instrument to a narrow mass range for the target molecule.
With HRMS methods, analytical chemists can easily perform qualitative and quantitative analysis for complex samples by first using a non-target full scan analysis to profile the multiple components in the sample and then use a targeted single ion monitor scan (SIM) to quantify each component of interest. HRMS is not only easier and more straightforward to use but also has comparable sensitivity to the LC-MS/MS method49.
A recent example utilised HRMS for the metabolomics study of complex biological matrices of the Panc-1 cell line as reported by Z. Wu et al.50. The group developed a HRMS full scan method for the analysis of non-targeted profile that provided structure annotation of multiple components with high confidence and quantitation of the components with high sensitivity. They then used the targeted HRMS-SIM scan method to enable the quantitative analysis of targeted metabolites with good linearity and comparable sensitivity. Z. Zendonga et al. have reported using HRMS for quantitative and qualitative screening of toxins in complex marine samples41. In this case, the high mass resolution of the HRMS enabled detection of a compound that co-eluted with the toxin, demonstrating the benefit of HRMS to detect co-eluting compounds. These examples demonstrate the power of HRMS for integration of non-targeted profiling analysis with targeted quantitative analysis for complex samples.
Ion mobility mass spectrometry
Ion mobility spectrometry (IMS) in combination with mass spectrometry (IM-MS) is an emerging tool that utilises ion mobility separation to aid in the analysis of complex samples51-52. IMS separates and identifies ionised molecules in the gas phase based on their mobility in a drift tube. The migration time through the tube is attributed to different ions by their mass, charge, size and shape, which leads to the separation of different species53. The ion’s collision cross-section (CCS) is the area that gas molecules interact with ions and CCS is related to ions’ chemical structure and three-dimensional conformation. The most popular IMS system is travelling wave ion mobility (TWIM) that is readily integrated with commercial LC-MS instruments, configured as LC-IM-MS systems. There are a wide range of IM-MS applications reported in the literature, including monitor oligomer formation54; separation of steroid isomers55; structural elucidation of drug metabolites56; identification of specific and non-specific binding of small molecules to protein targets57; receptor-based high-throughput screening and identification58; and structure based proteomics59. This is just a short list of the wide variety of applications of IM-MS reported.
A recent example utilising ion mobility mass spectrometry to study the structural isomers of drug metabolites, as reported by E. Reading et al.56, demonstrated the benefit of IM-MS. In this study LC-MS alone was unable to differentiate between the structural isomers in the mixture, since the isomers have the same mass and fragment ions. They demonstrated that LC-ESI-TWIM-MS technology was able to distinguish structural isomers of drug metabolites and enabled them to propose structures for each isomer. Since the isomers have different gas phase conformations, IM-MS is able to separate and differentiate the isomers based on their differences in collision cross section data (CCS). This example showed that IM-MS technology provides good sensitivity and fast scanning with ion mobility separation, mass separation and ion detection.
Structure identification by LC-HRMS
Providing identification and characterisation of reaction impurities is a crucial function in drug discovery and drug development processes. Medicinal chemists use this information to troubleshoot chemical reactions and optimise synthetic routes; development scientists also use the information to assess the safety of the drug candidates. LC-MS has long played a key role in providing this information and, more recently, high-resolution UHPLC-HR-MS has become the method of choice60-63. With the latest orbital trap technologies, mass spectrometers are now capable of providing the high scan rates needed to synchronise with the fast separation and narrow chromatography peaks from UHPLC. For example, orbital trap MS/MS scan rates can be up to 20Hz with unmatched spectral quality. In addition, it has a high mass accuracy across a wide scan range, such as 5ppm mass accuracy on a dynamic range of 500064, which enables simple data acquisition that provides both accurate mass and elemental composition. These advances in resolution and sensitivity lead to more confident structural elucidation, especially when combined with MS/MS fragmentation ion scans.
To demonstrate the benefits of LC-HR-MS, we utilised LC-HR-MS to characterise the multiple impurities of fexofenadine (F9427-10MG, Sigma-Aldrich). The LC-HR-MS data for fexofenadine is shown in Figure 2 (page 00). With enough sample loading into the LC-MS system, the UV chromatogram at 220nm clearly indicates multiple impurities in this sample, labeled P1 to P9 (Figure 2A; page 00). The high resolution accurate mass spectra were easily acquired during the LC-HR-MS run. Figure 2B (page 00) shows P8 has observed m/z 458.3058 amu, which confirmed the formula of C31H40NO2 for impurity P8 with error of 0.8941 ppm. Figure 2C shows the corresponding MS/MS spectrum of impurity P8 and the resulting fragment ions. The chemical structures for most of the fragment ions have been proposed based on their elemental compositions and parent molecule structure, which leads to high confidence in the proposed structure of impurity P8. This is just one example of how LC-HR-MS/MS technology is an efficient tool for structural elucidation.
Summary
We have described a few of the latest LC-MS technologies and their application in small molecule drug discovery. Advancements have occurred not only with instrumentation and ionisation technology but also with separation science, such as multidimensional LC systems and SFC. The latest ionisation technologies have expanded mass detection capabilities for analysing a wide variety of compounds. Multidimensional LC-MS utilises orthogonal separation and analysis for solving challenging problems. HRMS has become a straightforward and powerful tool for profiling and quantifying compounds of interest in complex mixtures. The continuing evolution in mass spectrometers and separation science will only increase the power and application of LC-MS in all areas of research.
References
- JP, Tees S, Kalindjian SB, Philpott KL. Principles of early drug discovery. Br J Clin Pharmacol. 2011; 162: 1239-1249
- Hoelder S, Clarke PA, Workman P. Discovery of small molecule cancer drugs: Successes, challenges and opportunities. Mol Oncol. 2012; 6: 155-176
- Schenone M, Dančík V, Wagner BK, Clemons PA. Target identification and mechanism of action in chemical biology and drug discovery. Nat Chem Biol. 2013; 9: 232–240
- Lam KS. New aspects of natural products in drug discovery. Trends in Microbio. 2007; 15(6): 289-299
- Ganesan A. The impact of natural products upon modern drug discovery. Curr Opin Chem Biol. 2008; 12: 306-317
- Rishton GM. Natural products as a robust source of new drugs and drug leads: Past successes and present day issues. Am J Cardiol. 2008; 101(A): 43-49
- Raevsky OA. Physicochemical descriptors in property-based drug design. Mini-Rev. Med Chem. 2004; 4: 1041-1052
- Hajduk PJ, Greer J. A decade of fragment-based drug design: strategic advances and lessons learned. Nat Rev Drug Discov. 2007; 6:211-219
- Waterbeemd HV, Smith DA, Beaumont K, Walker DK. Property-based design: Optimization of drug absorption and pharmacokinetics. J Med Chem. 2001; 44(9): 1313-1333
- Hopkins AL. Network pharmacology: the next paradigm in drug discovery. Nat Chem Biol. 2008; 4(11): 682-690
- Lipinski CA. Drug-like properties and the causes of poor solubility and poor permeability.
- J Pharmacol Tox Met. 2000; 44: 235-249
- Kwok D. Applications of liquid chromatography mass spectrometry in accelerating the pace of lead-drug candidate selection and pre-clinical development. Drug Discov World. 2004; Fall: 35-42
- Korfmacher WA. Principles and applications of LC–MS in new drug discovery, Drug Discov Today. 2005; 10(20): 1357-1367
- Du Q, Huang R, Chou K. Recent advances in QSAR and their applications in predicting the activities of chemical molecules, peptides and proteins for drug design. Curr Protein Pept Sc. 2008; 9: 248-259
- Perkins R, Fang H, Tong W, Welsh WJ. Quantitative structure-activity relationship methods: Perspectives on drug discovery and toxicology. Environ Toxicol Chem. 2003; 22(8): 1666–1679
- Lipinski CA. Lead- and drug-like compounds: the rule-of-five revolution. Drug Discov Today: Technologies. 2004; 1(4):337-341
- Xu RN, Fan L, Rieser MJ, El-Shourbagy TA. Recent advances in high-throughput quantitative bioanalysis by LC-MS/MS. J Pharm Biomed Anal. 2007; 44: 342-355
- Gu C, et al. Mass spectrometry in small molecule drug development. Am Pharm Rev. 2015; 30:1-12
- Taylor T. Understanding Electron Ionization Processes for GC–MS. LCGC N Am. 2015; 33 (4): 290
- Xu X, Craats AM, Kok EM, Bruyn P. Trace Analysis of Peroxide Explosives by High Performance Liquid Chromatography-Atmospheric Pressure Chemical Ionization-Tandem Mass Spectrometry (HPLC-APCI-MS/MS) for Forensic Applications. J Forensic Sci. 2004; 49(6):1-7
- Grayer RJ, Kite GC, Abou-Zaid M, Archer LJ. The Application of Atmospheric Pressure Chemical Ionization Liquid Chromatography-Mass Spectrometry in the Chemotaxonomic Study of Flavonoids:
- Characterization of Flavonoids from Ocimum gratissimum var. gratissimum. Phytochem Anal. 2000; 11: 257-267
- Eldridge GR, Vervoort HC, Lee CM, Cremin PA, Williams CT, Hart SM, Goering MG, Johnson MO, Zeng L. High-throughput method for the production and analysis of large natural product libraries for drug discovery. Anal Chem. 2002; 74: 3963-3971
- Fortin DT, Chen R. Developing a trace level GC–MS method for detecting methylhydrazine in an experimental drug substance. J Chrom Sci. 2010; 48:299-302
- Boumrah Y, Rosset M, Lecompte Y, Bouanani S, Khimeche K, Dahmani A. Development of a targeted GC/MS screening method and validation of an HPLC/DAD quantification method for piperazines–amphetamines mixtures in seized material. Egyptian J of Forensic Sci. 2014; 4:90-100
- Nair H, Woo F, Hoofnagle AN, Baird GS. Clinical validation of a highly sensitive GC-MS platform for routine urine drug screening and real-time reporting of up to 212 Drugs. J Toxicol. 2013; 1-7
- Villamor JL, Bermejo AM, Fernandez P, Tabernero MJ. A new GC-MS method for the determination of five amphetamines in human hair. J Anal Toxicol. 2005; 29: 135-139
- Ebinger K, Weller HN, Kiplinger J, Lefebvre P. Evaluation of a new preparative supercritical fluid chromatography system for compound library purification: The TharSFC SFC-MS Prep-100 System, JALA. 2011; June: 241-249
- Wong M, Murphy B, Pease JH, Dong MW. Separation science in drug development, Part I: High-throughput purification. LCGC N Am. 2015; 33(6): 402-412
- Kott L. An overview of supercritical fluid chromatography mass spectrometry (SFCMS) in the pharmaceutical industry. Am Pharm Rev. 2013; Feb:1-9
- Chen R. A brief review of interfacing supercritical fluid chromatography with mass spectrometry. Chrom Today. 2009; February/March: 11-13
- Gross JH. Direct analysis in real time – a critical review on DART-MS. Anal Bioanal Chem. 2014; 406:63-80
- Li LP, Feng BS, Yang JW, Chang CL, Liu HW. Applications of ambient mass spectrometry in high-throughput screening. Analyst. 2013; 138: 3097-3103
- Alexander AJ, Ma L. Comprehensive two-dimensional liquid chromatography separations of pharmaceutical samples using dual Fused-Core columns in the 2nd dimension. J Chrom A. 2009; 1216: 1338-1345
- Zhang K, Wang J, Tsang M, Wigman L, Chetwyn NC. Two-dimensional HPLC in pharmaceutical analysis. Am Pharm Rev (Rev Am Pharm Bus Tech). 2013; 1-12
- Pursch M, Bucknmaier S. Loop-based multi-cutting two-dimensional liquid chromatography for target analysis in complex matrices. Anal Chem. 2015; 87:5310-5317
- Venkatramani CJ, Wigman L, Mistry K, Chetwyn N. Simultaneous, sequential quantitative achiral-chiral analysis by two-dimensional liquid chromatography. J Sep Sci. 2012; 35:1748-1754
- Bedani F, Schoenmaakers PJ, Janssen HG. Theories to support method development in comprehensive two-dimensional liquid chromatography – A review. J Sep Sci. 2012; 35:1697-1711
- Li Y, Gu C, Gruenhagen J, Zhang K, Yehl P, Chetwyn NP, Medley CD. A size exclusion-reversed phase two dimensional-liquid chromatography methodology for stability and small molecule related species in antibody drug conjugates. J Chrom A. 2015; 1393: 81-88
- Li Y, Hewitt D, Lentz YK, Ji JA, Zhang TY, Zhang K. Characterization and stability study of polysorbate 20 in therapeutic monoclonal antibody formulation by multidimensional ultrahigh-performance liquid chromatography−charged aerosol detection−mass spectrometry. Anal Chem. 2014; 86: 5150−5157
- Zhou Y, Wang Y, Wang R, Guo F, Yan C. Tow-dimensional liquid chromatography coupled with mass spectrometry for the analysis of Lobelia chinensis Lour. Using an ESI/APCI multimode ion source. J Sep Sci. 2008; 31(13): 2388-2394
- Conti M, Motta R, Puggioli C, Brambilla P. Surface-activated chemical ionization-electrospray ionization mass spectrometry combined with two-dimensional serial chromatography is a powerful tool for drug stability studies. Rapid Comm Mass Spec. 2013; 27(11):1231-1236
- Qu L, Xiao Y, Jia Z, Wang Z, Wang C, Hu T, Wu C, Zhang J. Comprehensive two-dimensional liquid chromatography coupled with quadrupole time-of-flight mass spectrometry for chemical constituents analysis of tripterygium glycosides tablets, J Chrom A. 2015; 1400:65-73
- Venkatramani CJ, Al-Sayah M, Li G, Goel M, Girotti J, Zhang L, Wigman L, Yehl P, Chetwyn N. Simultaneous achiral-chiral analysis of pharmaceutical compounds using two-dimensional reversed phase liquid chromatography-supercritical fluid chromatography. Talanta. 2016; 148:548-555
- Rauh M. LC-MS/MS for protein and peptide quantification in clinical chemistry. J Chrom B. 2012; 883-884:59-67
- Xu RN, Fan L, Rieser MJ, El-Shourbagy TA. Recent advances in high-throughput quantitative bioanalysis by LC–MS/MS. J Pharm Biomed Anal. 2007; 44(2) 342–355
- Merrill AH, Sullards MC, Allegood JC, Kelly S, Wang E. Sphingolipidomics: High-throughput, structure-specific, and quantitative analysis of sphingolipids by liquid chromatography tandem mass spectrometry. Methods. 2005; 36(2): 207–224
- Acena J, Stampachiacchiere S, Perez S , Barcelo D. Advances in liquid chromatography-high-resolution mass spectrometry for quantitative and qualitative environmental analysis. Anal Bioanal Chem. 2015; 407:6289-6299
- Hopfgartner G, Tonoli D, Varesio E. High resolution mass spectrometry for integrated qualitative and quantitative analysis of pharmaceuticals in biological matrices. Anal Bioanal Chem. 2012; 402: 2587-2596
- Zendong Z, McCarron P, Herrenknecht C, Sibat M, Amzil Z, Cole RB, Hess P. High resolution mass spectrometry for quantitative analysis and untargeted screening of algal toxins in mussels and passive samples. J Chrom A. 2015; 1416: 10-21
- Wu Z, Bi H. Metabolomics studies paradigm shift with quanfirmation: Integrating untargeted profiling, targeted, and pseudo-targeted analysis on one platform. 2015; Am Soc Mass Spec Conf. Preceding
- Kanu AB, Dwivedi P, Tam M, Matz L, Hill Jr HH. Ion mobility-mass spectrometry. J Mass Spec. 2008; 43:1-22
- Guevremont R. High-field asymmetric waveform ion mobility spectrometry: A new tool for mass spectrometry, J Chrom A. 2004; 1058:3-19
- Creaser CS, Griffithe JR, Bramwell CJ, Noreen S, Hill CA, Thomas CLP. Ion mobility spectrometry: a review. Part 1. Structural analysis by mobility measurement. Analyst, 2004;129:984-994
- Young LM, Cao P, Raleigh DP, Ashcroft AE, Radford SE. Ion mobility spectrometry-mass spectrometry defines the oligomeric intermediates in amylin amyloid formation and the mode of action inhibitors, J Am Chem Soc. 2014; 136:660-670
- Ahonen L, Fasciotti M, Gennas GB, Kotiaho T, Daroda RJ, Eberlin M, Kostiainen R. Separation of steroid isomers by ion mobility mass spectrometry, J Chrom A. 2013; 1310:133-137
- Reading E, Munoz-Muriedas J, Roberts AD, Dear GJ, Robison CV, Beaumont C. Elucidation of drug metabolite structural isomers using molecular modeling coupled with ion mobility mass spectrometry. Anal Chem. 2016; 88:2273-2280
- Young LM, Saunders JC, Mahood RA, Revill CH, Foster RJ, Tu LH, et al. Screening and classifying small-molecule inhibitors of amyloid formation using ion mobility spectrometry-mass spectrometry. Nat Chem. 2014; 7:73-81
- Aqai P, Blesa NG, Major H, Pedotti M, Varani L, Ferroro VEV, et al. Receptor-based high-throughput screening and identification of estrogens in dietary supplements using bioaffinity liquid-chromatography ion mobility mass spectrometry. Anal Bioanal Chem. 2013; 405:9427-9436
- Zhong Y, Hyung KJ, Ruotolo BT. Ion mobility-mass spectrometry for structural proteomics. Expert Rev Proteomics. 2012; 9(1):46-58
- Barbara JE, Kazmi F, Muranjan S, Toren PC, Parkinson A. High-resolution mass spectrometry elucidates metabonate (false metabolite) formation from alkylamine drugs during In vitro metabolite profiling. Drug Metab Dispos. 2012; 40: 1966-1975
- Chen G, Pramanik BN, Liu YH, Mirza UA. Applications of LC/MS in structure identifications of small molecules and proteins in drug discovery. J Mass Spectrom. 2007; 42:279-287
- Kind T, Fiehn O. Advances in structure elucidation of small molecules using mass spectrometry. Bioanal Rev. 2010; 2:23–60
- Chen G, Pramanik BN. Application of LC/MS to proteomics studies: current status and future prospects. Drug Discov Today. 2009; 14 (9/10): 465-471
- Makarov A, Denisov E, Lange O, Horning S. Dynamic range of mass accuracy in LTQ orbitrap hybrid mass spectrometer. J Am Soc Mass Spectrom. 2006; 17:977-982
Biographies
Baiwei Lin is a Scientist in the Analytical Chemistry/Purification department in Small Molecule Discovery Chemistry at Genentech. Dr. Lin joined Genentech in 2007 and developed methods for high throughput QC, characterisation, quantification, and physicochemical property determinations. Prior to Genentech, she was a scientist at Berlex Biosciences and specialised in LCMS method development for characterisation of small molecules, peptides and proteins. Baiwei earned a MS degree in Chemical Engineering and a PhD in Analytical Chemistry from Montana State University, USA.
Christine Gu is currently a scientist in the Department of Small Molecule Pharmaceutical Science at Genentech. She is a subject matter expert in mass spectrometry. Before joining Genentech, she worked as a senior application scientist at ThermoFisher Scientific. She holds a PhD degree in Toxicology at University of California, Riverside.
Kate Comstock is a Senior Marketing specialist in Pharma and Biopharma Marketing at Thermo Fisher Scientific to support new product development and small molecule applications in the pharmaceutical industry using high resolution mass spectrometry. Prior to Thermo, Kate was a research scientist at Roche Pharmaceutical for 20 years, where she supported drug R&D and production using a variety of LCMS systems for API impurity ID and structure elucidation. Kate has a BS in chemistry and MS in pharmaceutical sciences from China and MS in Chemistry from San Jose State University, USA.
Joseph Pease, PhD, is Principal Scientific Manager and head of the Analytical Chemistry & Purification department in Small Molecule Discovery Chemistry at Genentech. Dr. Pease earned a BS degree in Chemistry from the University of California, Davis and a PhD in Biophysical Chemistry from the University of California, Berkeley. He started his career at Syntex (later Roche Palo Alto) as a Research Scientist specialising in NMR and structure-based drug discovery. He stayed with the company until its closure in 2010 and joined Genentech in 2011. During his career, Dr. Pease has held leadership positions in research, preclinical development, and informatics.
Related topics
Analysis, Mass Spectrometry
Related organisations
Genentech
Related people
Baiwei Lin, Chunang Gu, Joseph Pease, Kate Comstock