Nanoparticle-mediated enzyme delivery for application in cancer therapy
Posted: 9 March 2017 | Mukanth Vaidyanathan (University of California), Negin Mokhtari (University of California), Sadik Esener (University of California), Ya-San Yeh (University of California) | No comments yet
Enzyme therapy is a promising form of cancer treatment. The specific nature of enzyme and substrate interaction gives enzyme therapy an edge compared to standard non-specific therapies such as radiation and chemotherapy. However, since most of these enzymes are of a foreign nature, the delivery of these immunogenic enzymes has been a challenge. This study shows that enzyme encapsulation in nanoparticles may shield them from the immune system, allowing for the potential of targeted delivery and reduction of off-target adverse side effects.
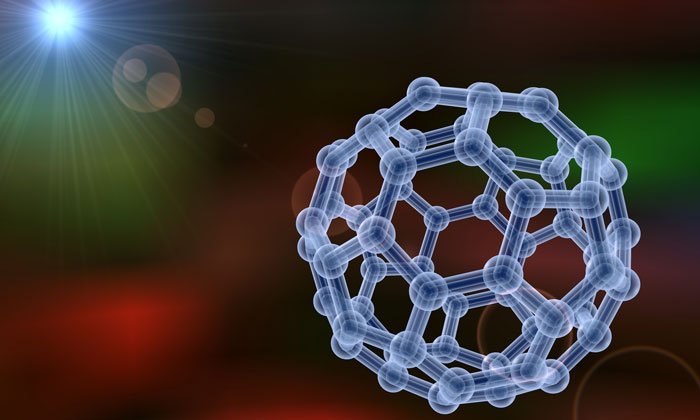
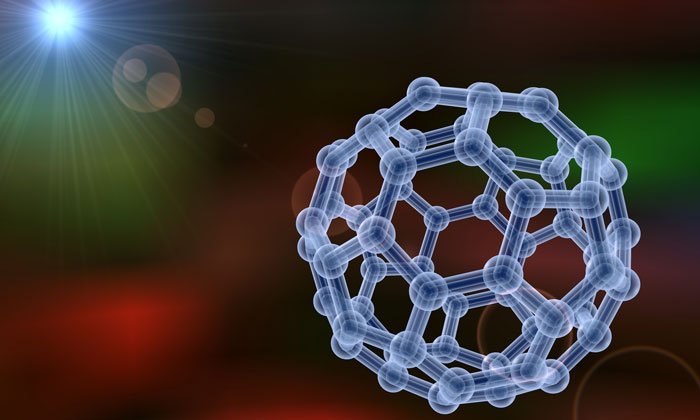
In 2012, 14.1 million patients were diagnosed with cancer, of which 8.2 million cases were fatal. This number is expected to rise to 19.3 million new cancer cases by 2025.1 There are several therapy techniques available to treat cancer, but with limited success. Chemotherapy (either single or in combination), surgery and radiation therapy have not been successful in improving the survival of patients, despite tumour response having been favourable.2,3 Success rates of 15-20% have been observed with immunotherapy. Even with these advanced treatments available commercially, newer methods are still required to improve the survival and quality of life of the patient. One effective method for the treatment of cancer is amino acid depletion therapy.
Asparaginase and arginine deaminase lower non-essential amino acid asparagine and arginine, respectively, in circulation. Asparaginase is effective for the treatment of acute lymphoblastic leukaemia,4 and arginine deaminase for treating melanoma and hepatocellular carcinoma.5 Hence, there is a priority in amino acid depletion therapy for the treatment of cancer. Moreover, many of the potent cancer drugs are also toxic to normal cells. To overcome the systemic toxicity of cancer drugs, a non-toxic pro-drug form of the potent drug can be activated with an enzyme at the tumour site.6 Unfortunately, most of these enzymes have a non-human origin, which generates immense antibody neutralisation and rapid clearance from the body.5
Currently there are two approaches to prevent an immune response against foreign enzymes and maintain the enzyme activity. First, the enzyme is modified directly, for instance with polyethylene glycol (PEG), which may cause partial loss of enzymatic activity and stability and in some cases generate immune response against PEG.7 As a result, enzyme modifications are met with limited success of protecting the enzyme from immune clearance. Second, is thorough encapsulation of modified or unmodified enzyme by organic/inorganic nanoparticles.8 The second method promises low-cost production and increased homogeneity.9
These enzymes are encapsulated or immobilised by a nanoparticle delivery vehicle, which can either be released or remain at the site of injection and allow for enzyme-substrate interaction.10
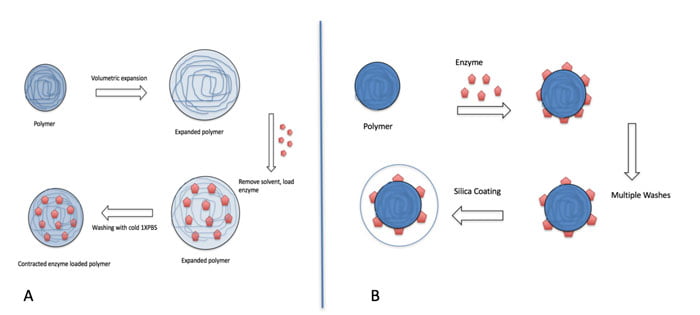
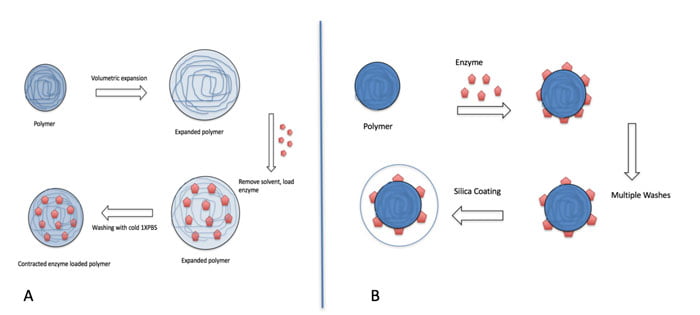
Figure 1: Schematic of two methods of enzyme encapsulation with polymeric nanoparticles.(A) Volumetric expansion and contraction of polymeric nanoparticles to encapsulate enzymes. (B) Coating of enzyme adsorbed polymeric nanoparticles to encapsulate enzymes.
The nanoparticles could potentially be used for local, circulatory, and intracellular applications. Moreover, the nanoparticles could allow for targeted therapy. The combination of targeting nanoparticles and enzyme therapy (that is very specific in nature), will further minimise side effects and damage to healthy organs.
Nanoparticles could be used in both passive and active targeting. The enhanced permeation and retention effect of the leaky tumour vasculature could allow for passive targeting of nanoparticles to the tumours.^11 In addition, the surface of the nanoparticle can be designed with targeting ligands, such as vascular endothelial growth factor (VEGF) that can be actively targeted to specific VEGF receptors in the vascular endothelium. However, particularly in the case of payloads like enzymes, due to the relatively large size of some enzymes and their low stability, encapsulation of enzymes and immobilisation with nanoparticles has had limited success in the past.
Encapsulating immunogenic enzymes
Two widely studied methods of encapsulating immunogenic enzymes with polymeric nanoparticles are investigated in this study. Polymers are used as a scaffold to immobilise enzymes. The nanoparticles can subsequently be sealed with a coating such as a porous silica layer. The first enzyme encapsulation method uses volumetric expansion and contraction of polymeric nanoparticles to encapsulate enzymes, thereby shielding the enzymes from the immune system.
Polymeric nanoparticles are first exposed to solvents such as ethanol, acetonitrile, or ether, that cause the spacing between the polymers to increase through polar interactions,^12 thereby expanding the nanoparticles and increasing the pore size. The solvents are then removed with multiple washes with water before adding enzymes to the expanded nanoparticles. With the pores enlarged, enzymes are able to diffuse within the nanoparticles. The nanoparticles are contracted by reducing the temperature. Figure 1(A) shows a schematic of the expansion and contraction process of encapsulating enzymes in polymeric nanoparticles.
The second enzyme encapsulation method uses hydrophobic-hydrophilic interaction to adsorb the enzymes onto polymeric nanoparticles,^13 then shield the enzymes from the immune system with a biocompatible coating, such as silica or calcium phosphate.^14 Figure 1(B) shows a schematic of the adsorption and coating process of encapsulating enzymes in polymeric nanoparticles. Since in the immobilised enzyme-polymer complex the foreign enzyme will still be exposed to the immune system, further protection of the complex could assist with a more complete protection and reduced immune response in vivo.
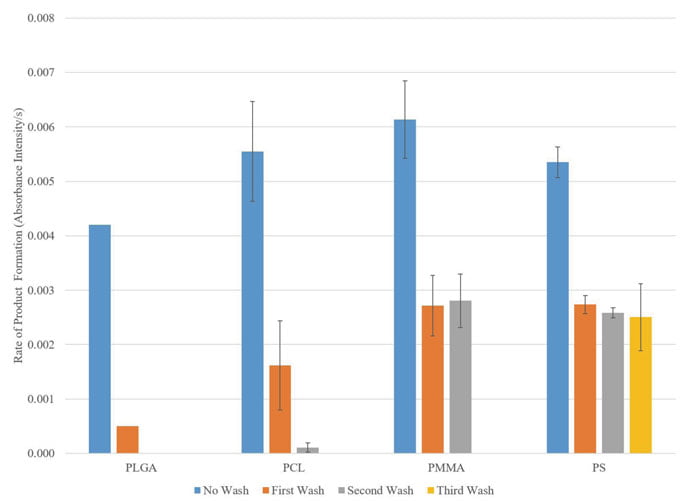
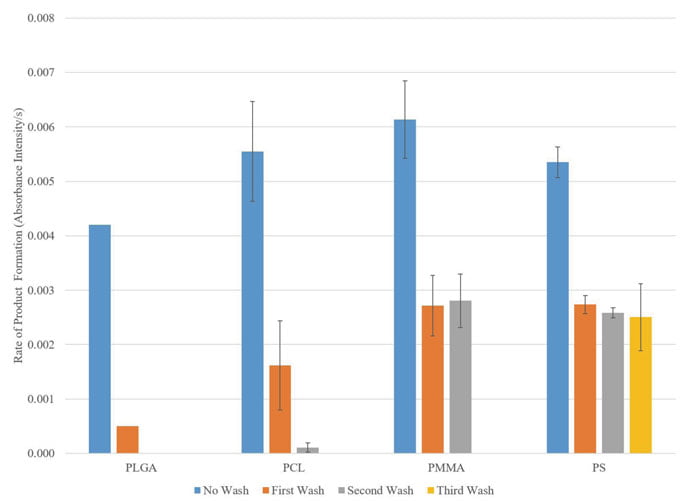
Figure 2: Adsorption of beta-lactamase enzymes onto PLGA, PCL, PMMA, and PS nanoparticles. The enzymatic activity of beta-lactamase is determined by the rate of hydrolysation of the amide bond in the beta-lactam ring by the enzyme, which is measureable in the change in absorbance intensity.
For this purpose, silica was selected as the coating material. Silica is an excellent candidate due to its biodegradability, biocompatibility, low toxicity, and adjustable porosity, as well as thermal and mechanical stability, making it suitable for in vivo applications.^15-18 The method described here allows for the encapsulation of an enzyme without modifying the enzyme itself. The small size and inert material used in the fabrication of these nanoparticles could allow for an enhanced circulation half life, while the surface of the nanoparticle allows for potential specific targeting agents. The porous nature of the silica coating is big enough for small molecules like the substrate and the resulting product to diffuse in and out of the nanoparticles, but not large enough for the enzymes to escape, and therefore the enzymes are protected within the nanoparticles.
Method and results
In this study biocompatible and biodegradable polymers^19-21 were selected for evaluation of enzyme encapsulation via the two methods described previously. Four 200nm polymeric nanoparticles that are commercially available were purchased for testing: poly(lactic-co-glycolic acid) (PLGA), polycaprolactam (PCL), poly(methyl methacrylate) (PMMA), and polystyrene (PS). The enzyme beta-lactamase was used as a substitute enzyme in this experiment. Enzyme activity assay was performed using nitrocefin as the substrate. Nitrocefin is a chromogenic beta-lactamase substrate that changes colour from yellow (λmax = 390 nm at pH 7.0) to red (λmax = 486 nm at pH 7.0) as the amide bond in the beta-lactam ring is hydrolysed by the enzyme.
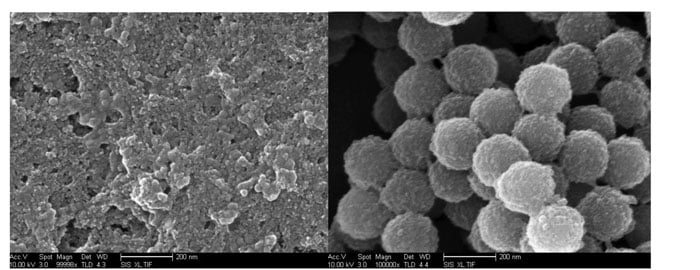
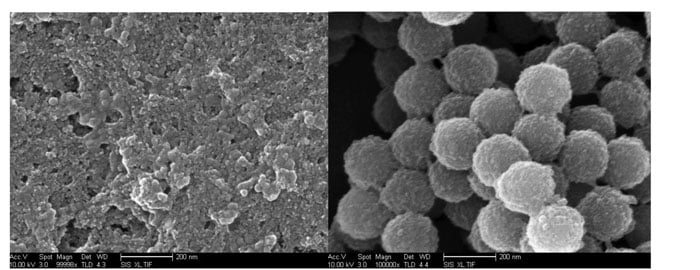
Figure 3: Scanning electron microscope image of uncoated 200-nm PMMA nanoparticles
degraded after three weeks (left) and silica coated and betalactamase adsorbed 200-nm polystyrene nanoparticles (right).
Polymers have the tendency to increase or decrease in size due to many physical parameters such as temperature, solvent, pH and other external stimuli (light, ultrasound).^22 Three solvents – acetonitrile, diethyl ether, and ethanol – were used to study the nanoparticle expansion. The nanoparticles were incubated at room temperature in respective solvents for 10, 15, and 30 minutes, before being measured in the respective dispersed solvents. Next, the polymeric nanoparticles were washed with phosphate buffered saline (PBS) three times to remove the organic solvent. Concentrated enzyme solution was incubated with the nanoparticles overnight while stirring at 4C to ensure gradual contraction of the polymeric nanoparticle. The nanoparticles were washed with ice cold PBS three times and the supernatant and pellet was collected after every wash. The activity was evaluated with nitrocefin assay.
No significant activity was detected with any polymeric nanoparticle incubated with either of the three organic solvents. It is possible that the pore size of the particle was not large enough for the enzyme to enter the nanoparticle, or the size of the pores was too large even after contraction for the enzyme to remain encapsulated. The method of enlargement of the nanoparticle involves the use of organic solvents, which may be an unfavourable medium for the enzyme to remain active. In future, protection of the enzymatic active site can be achieved by enzyme stabilising agents (eg, albumin, glucose, sucrose) in the presence of organic solvent.
The adsorption and coating processes for enzyme encapsulation were evaluated in two steps. To evaluate the adsorption process, each type of polymeric nanoparticle was incubated with beta-lactamase overnight for the enzymes to adsorb onto the nanoparticles. The enzyme adsorption was challenged by washing the enzyme adsorbed nanoparticles multiple times and testing the enzymatic activity after each wash. Based on the results shown in Figure 2, the enzyme adsorption onto PLGA and PCL nanoparticles was not strong enough to withstand more than three washes, as demonstrated by the drop in enzymatic activity.
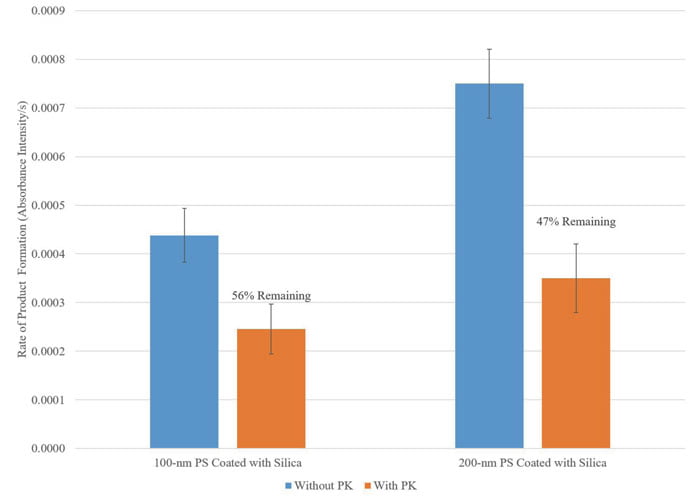
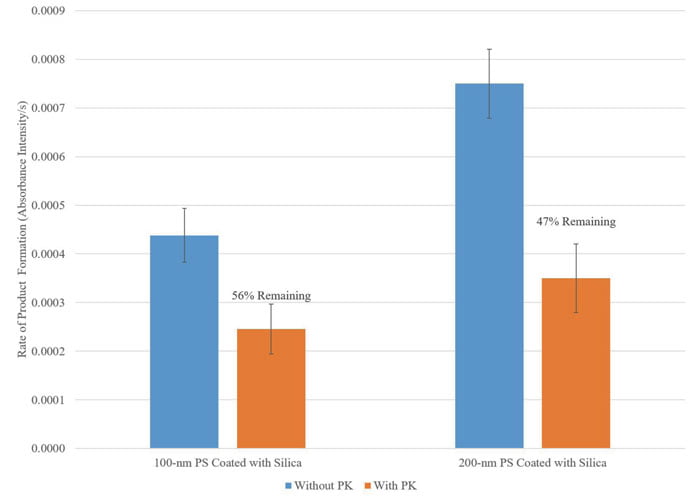
Figure 4: Nanoparticle encapsulated betalactamase were incubated with PK at 37°C in a shaking incubator overnight. Enzyme activity was measured using nitrocefin assay.
The enzyme adsorption onto PMMA and PS nanoparticles were stable for at least two additional washes after the initial free enzymes were washed away. However, the PMMA nanoparticles were not stable in solution for an extended period of time. The scanning electron microscope image in Figure 3 (left) showed the degradation of the 200nm PMMA nanoparticles after three weeks in PBS. The PS nanoparticles were stable in solution after three weeks (images not shown). Based on these findings, enzyme adsorbed PS nanoparticles are selected for the subsequent coating process.
In order to coat the enzyme-PS nanoparticles with the porous silica layer, poly-L-lysine (PLL) was added to the enzyme-PS mixture in PBS. Silica sol-gel was precipitated on the enzyme-PS mixture using silicic acid as a precursor and incubated at 4C overnight whilst being stirred. The subsequent mixture was washed multiple times to remove any unreacted reagents. A scanning electron microscope image of the final silica coated PS nanoparticles is shown in Figure 3 (right).
To further challenge the protective capability of the silica-coated nanoparticles, the silica-coated PS nanoparticles were incubated with proteinase K. Proteinase K (PK) is a serine protease that will cleave any free enzyme that is not encapsulated within the nanoparticles or any enzyme that is on the surface. Beta-lactamase unencapsulated and encapsulated within PS nanoparticles were incubated with PK at 37C in a shaking incubator overnight. Enzymatic activity post PK incubation was measured using the nitrocefin assay.
Unencapsulated beta-lactamase lost all of its activity upon exposure to PK (not shown), while nanoparticle encapsulated beta-lactamase was able to retain more than 50% of its activity as shown in Figure 4. The partial loss of activity in nanoparticles can be due to free enzymes already present in the nanoparticle solution due to incomplete washing, as well as loss of activity from the portion of the enzymes that are immobilised close to the silica surface. The sustained activity of the enzymes encapsulated in the nanoparticles post PK exposure is a demonstration of the protection ability of the said nanoparticle of the enzyme against proteolysis in vivo.
Summary
In conclusion, this study found that enzyme adsorbed onto polystyrene nanoparticles and encapsulated with silica coating has the protective capability of the encapsulated enzymes while challenged by extreme protease degradation. Future improvements to enzyme encapsulation in nanoparticles may include the shift from solid core polymeric nanoparticles to hollow polymeric nanoparticles to possibly increase the amount of enzyme encapsulation and decrease the polymeric material content. In addition, the nanoparticle materials can be expanded to include lipids, proteins, silica, and calcium phosphate.
Biographies
MUKANTH VAIDYANATHAN is a chemical engineering PhD candidate at the University of California, San Diego (UCSD). His research focuses on enzyme/protein delivery using nanoparticles for diagnostic and therapeutic applications in the brain and targeted oxidative therapy for treatment in cancer.
YA-SAN YEH is a bioengineering PhD graduate student at the University of California San Diego, with specialisation in multi-scale biology. Her research focus is on the fabrication of silica nanoparticles for enzyme and drug delivery in cancer applications.
PROF SADIK ESENER is the Director for Cancer Early Detection and Prevention Center at Oregon Health and Sciences University (OHSU) and a Professor of Nanoengineering and Electrical Engineering at UCSD. His current focus is on cancer early detection and prevention as well as therapeutics and diagnostics application of enzyme, virus, and drug-loaded nanoparticles.
References
- Center For Disease Control And Prevention: Global cancer statistics, 2015 Feb 2. cdc.gov/cancer/international/statistics.htm
- Heinemann V, Boeck S, Hinke A, Labianca R, Louvet C. Meta-analysis of randomized trials: evaluation of benefit from gemcitabine-based combination chemotherapy applied in advanced pancreatic cancer. BMC cancer. 2008 Mar 28;8(1): 82
- Fujiki T, Futatsuki K, Akazawa S, Yamamoto K, Kanda Y, Yamato A, Terashi K, Suda Y. Ifosfamide chemotherapy ineffective for advanced pancreatic carcinoma. Gan to kagaku ryoho. Cancer & chemotherapy. 1997 Mar;24(5): 569-72
- Pieters R, Hunger SP, Boos J, Rizzari C, Silverman L, Baruchel A, Goekbuget N, Schrappe M, Pui CH. L-asparaginase treatment in acute lymphoblastic leukemia. Cancer. 2011 Jan 15;117(2): 238-49
- Feun L, You M, Wu CJ, Kuo MT, Wangpaichitr M, Spector S, Savaraj N. Arginine deprivation as a targeted therapy for cancer. Current pharmaceutical design. 2008 Apr 1;14(11): 1049-57
- Danks MK, Yoon KJ, Bush RA, Remack JS, Wierdl M, Tsurkan L, Kim SU, Garcia E, Metz MZ, Najbauer J, Potter PM. Tumor-targeted enzyme/prodrug therapy mediates long-term disease-free survival of mice bearing disseminated neuroblastoma. Cancer research. 2007 Jan 1;67(1): 22-5
- Veronese FM. Peptide and protein PEGylation: a review of problems and solutions. Biomaterials. 2001 Mar 1;22(5): 405-17
- Wang Y, Caruso F. Mesoporous silica spheres as supports for enzyme immobilization and encapsulation. Chemistry of Materials. 2005 Mar 8;17(5): 953-61
- Slowing II, Vivero-Escoto JL, Wu CW, Lin VS. Mesoporous silica nanoparticles as controlled release drug delivery and gene transfection carriers. Advanced drug delivery reviews. 2008 Aug 17;60(11): 1278-88
- Volodkin DV, Petrov AI, Prevot M, Sukhorukov GB. Matrix polyelectrolyte microcapsules: new system for macromolecule encapsulation. Langmuir. 2004 Apr 13;20(8): 3398-406
- Prabhakar U, Maeda H, Jain RK, Sevick-Muraca EM, Zamboni W, Farokhzad OC, Barry ST, Gabizon A, Grodzinski P, Blakey DC. Challenges and key considerations of the enhanced permeability and retention effect for nanomedicine drug delivery in oncology
- Fowkes FM, Tischler DO, Wolfe JA, Lannigan LA, Ademu-John CM, Halliwell MJ. Acid–base complexes of polymers. Journal of Polymer Science: Polymer Chemistry Edition. 1984 Mar 1;22(3): 547-66
- Soppimath KS, Aminabhavi TM, Kulkarni AR, Rudzinski WE. Biodegradable polymeric nanoparticles as drug delivery devices. Journal of controlled release. 2001 Jan 29;70(1): 1-20
- Tan W, Wang K, He X, Zhao XJ, Drake T, Wang L, Bagwe RP. Bionanotechnology based on silica nanoparticles. Medicinal research reviews. 2004 Sep 1;24(5):621-38
- Radin S, Falaize S, Lee MH, Ducheyne P. In vitro bioactivity and degradation behavior of silica xerogels intended as controlled release materials. Biomaterials. 2002 Aug 31;23(15):3113-22
- Shahbazi MA, Herranz B, Santos HA. Nanostructured porous Si-based nanoparticles for targeted drug delivery. Biomatter. 2012 Oct 1;2(4): 296-312
- Slowing II, Vivero-Escoto JL, Wu CW, Lin VS. Mesoporous silica nanoparticles as controlled release drug delivery and gene transfection carriers. Advanced drug delivery reviews. 2008 Aug 17;60(11): 1278-88
- Rosenholm JM, Mamaeva V, Sahlgren C, Lindén M. Nanoparticles in targeted cancer therapy: mesoporous silica nanoparticles entering preclinical development stage. Nanomedicine. 2012 Jan;7(1): 111-20
- Son SR, Linh NT, Yang HM, Lee BT. In vitro and in vivo evaluation of electrospun PCL/PMMA fibrous scaffolds for bone regeneration. Science and Technology of Advanced Materials. 2013 Mar 7;14(1): 015009
- El Fray M, Prowans P, Puskas JE, Altstädt V. Biocompatibility and Fatigue Properties of Polystyrene− Polyisobutylene− Polystyrene, an Emerging Thermoplastic Elastomeric Biomaterial. Biomacromolecules. 2006 Mar 13;7(3): 844-50
- Anderson JM, Shive MS. Biodegradation and biocompatibility of PLA and PLGA microspheres. Advanced drug delivery reviews. 2012 Dec 31;64: 72-82
- Liechty WB, Kryscio DR, Slaughter BV, Peppas NA. Polymers for drug delivery systems. Annual review of chemical and biomolecular engineering. 2010 Jul 15;1 :149-73
Related topics
Enzymes, Nanomedicine, Nanoparticles, Nanotechnology
Related conditions
Acute lymphoblastic leukaemia